Nuclear Charge: The Heartbeat of Every Atom and Sustainable Development
- bpsinghamu
- Apr 7
- 8 min read
A Journey Through History : At the dawn of the 20th century, the structure of the atom was still shrouded in mystery. Scientists imagined it as a "plum pudding" — a diffuse positive soup with embedded electrons, as J. J. Thomson proposed. But in 1909, something revolutionary happened. Under the supervision of Ernest Rutherford at the University of Manchester, his research assistant Hans Geiger and undergraduate student Ernest Marsden carried out a groundbreaking experiment, popularly known as the "gold foil experiment." In this experiment, they directed a beam of alpha particles at an extremely thin sheet of gold foil, expecting the particles to pass through with minimal deflection. To their astonishment, while most of the alpha particles did pass through, some were deflected at large angles — and a few even bounced straight back.
To detect these alpha particles, a circular zinc sulfide screen was placed around the gold foil in a movable microscope setup, allowing observation at various angles. When an alpha particle struck the screen, it produced a tiny flash of light (scintillation), which was manually counted by the experimenters through a microscope in a darkened room.
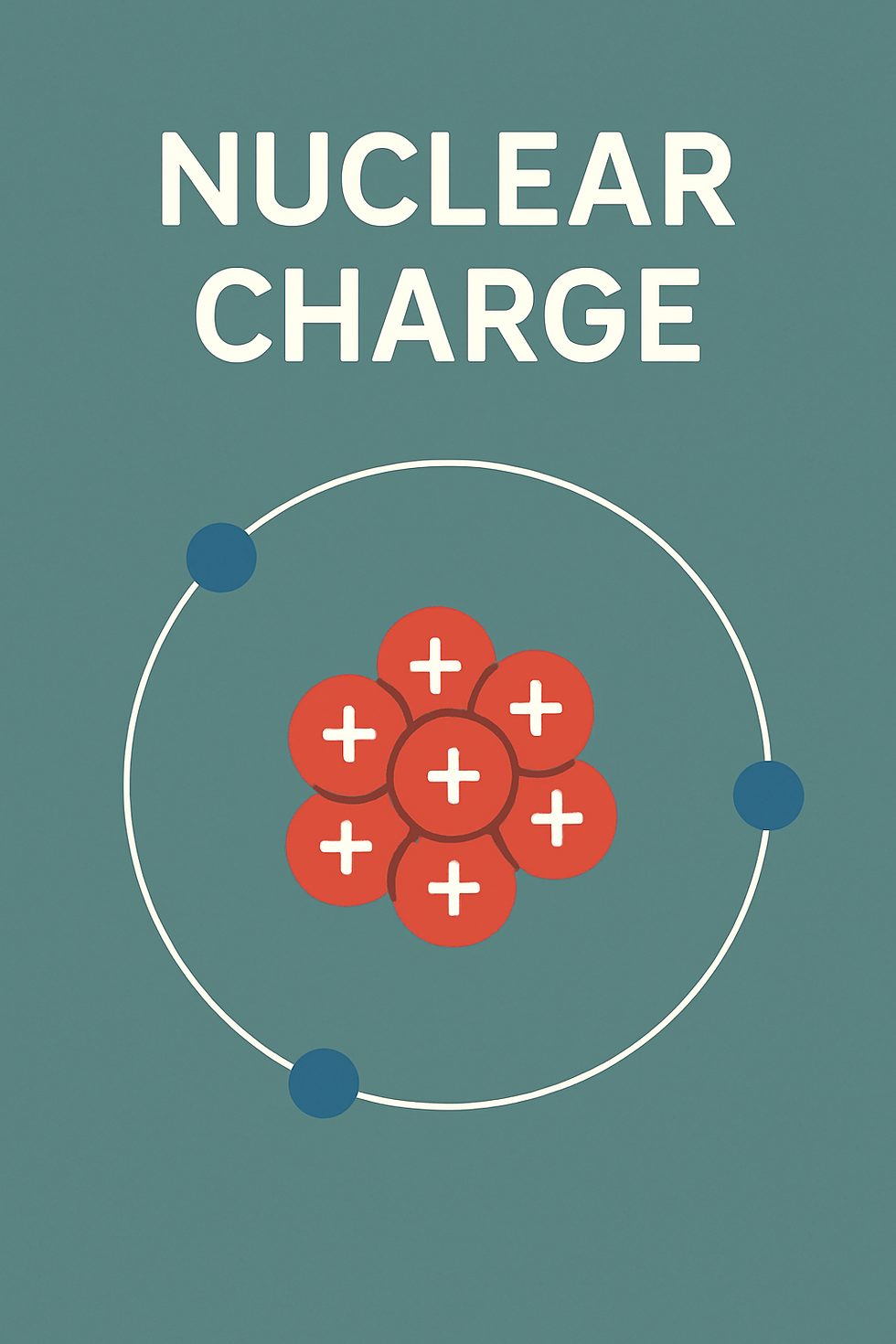
When Rutherford analyzed these unexpected results, he concluded that the atom must consist mostly of empty space, with a dense, positively charged core at the center — the nucleus. This marked a fundamental shift in our understanding of atomic structure. The nucleus, containing nearly all the atom’s mass and positive charge, became central to the concept of nuclear charge — the very identity of an element.
What is Nuclear Charge?
Nuclear charge is the total positive charge within the nucleus, determined by the number of protons (Z) it contains. Each proton carries a charge of +e = 1.602 × 10⁻¹⁹ C.Thus, for an element with Z protons, the nuclear charge is Z·e. Let us take example of Oxygen (Z = 8) — its nuclear charge is 8 × 1.602 × 10⁻¹⁹ C ≈ 1.28 × 10⁻¹⁸ Coulomb. This charge gives rise to the attractive force pulling electrons toward the nucleus, much like the Sun's gravitational pull keeps planets in orbit. Without this pull, the atom would simply fall apart, and would not be stable.
Real-Life Analogy: A COUNTRY and Its Prime Minister
Imagine a developing country. The nucleus is the Prime Minister (PM), and the electrons are the citizens. A strong and charismatic PM (high nuclear charge) keeps the country organized, the citizens loyal, and services running smoothly. But if the PM is weak or distracted (low Z), the country falls into chaos — the citizens scatter, and harmony is lost. Similarly, atoms with low nuclear charge hold electrons loosely, making them more chemically reactive or easier to ionize.
This is why sodium (Z = 11), with its single loosely bound outer electron, readily gives it up — making it crucial in life processes and energy transfer in your nerves! Let me elaboarate it: This is why sodium (Z = 11), which has just one electron in its outermost shell, behaves the way it does. That single outer electron is weakly bound compared to the electrons closer to the nucleus, making it easy for sodium to lose it and achieve a stable, noble gas-like configuration. When it gives up this electron, it becomes a positively charged ion (Na⁺), a form in which it plays a vital role in biological systems. In your body, sodium ions are essential for maintaining fluid balance, transmitting nerve impulses, and enabling muscle contractions. Every time a nerve signal travels through your body, it relies on the controlled movement of sodium (and potassium) ions across cell membranes — a fundamental process that powers communication between cells and keeps your body functioning smoothly.
Effective Nuclear Charge — The Real Pull
But here’s the twist: in atoms with multiple electrons, the inner electrons shield the outer ones from the complete pull of the nucleus. This gives rise to the concept of effective nuclear charge (Z_eff), which is calculated as: Z_eff = Z − σ, where σ is the shielding constant.
For example, in a sodium (Na) atom, there are 11 protons in the nucleus, each carrying a positive charge equal to 1.602 × 10⁻¹⁹ C. However, sodium also has 11 electrons arranged in shells around the nucleus. Ten of these electrons are located in the inner shells (two in the first shell and eight in the second), where they effectively "shield" or block the positive charge of the nucleus from reaching the 11th, outermost electron in the third shell. Because of this shielding effect, the outermost electron doesn’t feel the full attractive force of the +11e nuclear charge. Instead, it experiences an effective nuclear charge that is significantly less — closer to +1e.
This reduced attraction makes it much easier for sodium to lose that outer electron. When it does, it becomes a positively charged ion (Na⁺), achieving a stable electron configuration similar to neon, a noble gas. This tendency to lose its outer electron easily makes sodium highly reactive, especially with elements like chlorine, which readily accept electrons. The result? Common compounds like sodium chloride — table salt.
But sodium ions (Na⁺) do far more than just making food taste salty. As already mentioned, they are essential for life itself. In the human body, sodium ions help regulate fluid balance, generate electrical signals in neurons, and maintain the proper functioning of muscles and nerves. The rapid movement of sodium ions across cell membranes is a key part of how nerve impulses are generated and transmitted — a process fundamental to everything from thinking and feeling to moving your muscles.
Real-Life Resemblance: Parking Garage Shielding
Think of a multi-level parking garage. The nucleus is at the basement, while cars (electrons) are parked on different levels. The cars on the top floor can’t see or feel what’s happening in the basement because the floors in between block their view — just as inner electrons shield the outer ones from the nucleus. This shielding affects how strongly atoms hold on to their electrons and plays a vital role in determining reactivity, electronegativity, and ionization energy.
Periodic Trends and Atomic Behavior
The concept of nuclear charge explains why the periodic table behaves the way it does:
Atomic size decreases across a period as Z increases. More protons pull electrons closer.
Ionization energy increases with Z — more energy is needed to remove tightly held electrons.
Electronegativity rises with Z — atoms with higher nuclear charge attract bonding electrons more strongly.
This is why fluorine (Z = 9) is incredibly electronegative and highly reactive. With 9 protons in its nucleus and only one electron short of a full outer shell, fluorine exerts a strong pull on electrons from other atoms to complete its octet. The electrons are also relatively close to the nucleus, so the attractive force between the nucleus and incoming electrons is strong. This intense desire to gain an electron makes fluorine the most electronegative element in the periodic table. As a result, it readily forms compounds — often violently — and is found in substances like fluoride toothpaste and Teflon coatings due to its chemical strength and stability once it bonds.
On the other hand, potassium (Z = 19) behaves quite differently. With one loosely bound electron in its fourth shell, far from the attractive pull of its 19 protons (which are partially shielded by 18 inner electrons), potassium readily gives up that outer electron to achieve a stable, noble gas configuration like argon. This makes potassium highly reactive, especially with water and nonmetals like chlorine. Its ability to easily form positive ions (K⁺) makes it incredibly useful — it’s a key ingredient in fertilizers, helping plants grow by regulating ion balance and enzyme activity. And in fireworks, potassium compounds (like potassium nitrate or potassium perchlorate) act as oxidizers, providing the oxygen needed for explosive combustion and brilliant color displays.
How Do We Measure Nuclear Charge?
One of the earliest techniques, the X-ray spectrum method, was developed by Henry Moseley, who used X-ray spectroscopy to show that X-ray frequencies increased with atomic number, leading to Moseley’s Law:
frequency ∝ (Z − σ)
Nowadays, advanced techniques like high-energy electron scattering and the use of particle accelerators allow scientists to probe the inner structure of atoms with remarkable precision. In this method, electrons are accelerated to extremely high energies — often in the range of hundreds of MeV — and directed toward a target made of atoms. Because electrons are fundamental point-like particles and interact via the electromagnetic force (not the strong nuclear force), they serve as clean, precise probes of the nuclear charge distribution. This approach was pioneered by Robert Hofstadter in the 1950s at Stanford University. Using a linear accelerator, Hofstadter bombarded atomic nuclei with high-energy electrons and carefully analyzed how these electrons scattered after interacting with the nucleus. By studying the scattering angles and energy losses, he was able to infer the size, shape, and charge distribution of various nuclei.
Hofstadter’s experiments revealed that nuclei are not point-like, but have a finite size and a well-defined internal structure. His work showed, for instance, that charge density is generally highest at the center of the nucleus and gradually decreases toward the edges — a finding that was crucial in confirming the nuclear shell model and in developing our modern understanding of nuclear structure. Thanks to Hofstadter’s pioneering contributions, high-energy electron scattering became one of the most powerful tools in nuclear physics. For his groundbreaking discoveries, he was awarded the Nobel Prize in Physics in 1961.
Today, this technique is still widely used — with even higher energy and precision — in large research facilities around the world, offering valuable insights into nuclear shapes, exotic isotopes, and the substructure of protons and neutrons.
Cosmic Connection: Stars and Heavy Elements
The story of nuclear charge isn't confined to Earth — it’s written across the stars as well. In the heart of the Sun, nuclear charge drives fusion — where hydrogen nuclei (Z = 1) fuse to form helium (Z = 2). As stars undergo aging, they create heavier and heavier elements, building up nuclear charge with each step. The carbon in your cells, the calcium in your bones, and the iron in your blood were all born in ancient stars — cosmic furnaces where nuclear charge guided creation itself. In essence, we are made of atoms forged by nuclear charge. We are made of star stuff.
Nuclear Charge helps in Sustainable Technology
This isn’t just an statement but — it’s powerful. Let me give you a few examples;
Processes like Nuclear Fission and Fusion provide clean energy
Nuclear charge enables fission in high-Z atoms like uranium (Z = 92). When uranium splits, it releases tremendous energy without carbon emissions. A small pellet of uranium can power a city for days. It’s a clean, efficient alternative to fossil fuels, critical for combating climate change. Future technologies aim to harness nuclear fusion — fusing small nuclei (like hydrogen) into larger ones. If successful, fusion could provide limitless, clean energy — again, thanks to our understanding of nuclear charge.
Healthcare and High-Z Elements
High-Z elements like iodine (Z = 53) are used in medical imaging and cancer therapy. They absorb radiation efficiently, making them ideal for diagnostics and treatment. Even in everyday life — from X-ray contrast agents to radiotherapy isotopes, and PET scans to radiopharmaceuticals — nuclear charge determines how atoms interact with radiation and the human body. The high Z-materials like lead (Z=82) is very much helpful for schiedling due to gamma nd X-rays, both in healthcare and otherwise.
Electronics and Efficient Design
Semiconductors like silicon (Z = 14) and germanium (Z = 32) power your computers, phones, and solar panels. The behavior of these atoms — how easily electrons move or are excited — depends on their nuclear charge. Even battery technology and green transportation rely on materials whose atomic properties are shaped by the number of protons in their nuclei.
Nuclear Charge and Sustainable Development
So, how does nuclear charge link to sustainability?
It drives clean energy systems (fission and fusion).
It enables medical diagnostics and treatments, improving public health.
It supports precision agriculture using radioisotopes to reduce waste and improve yields.
It empowers green electronics, smarter grids, and reduced energy consumption.
In short, nuclear charge is at the heart of every atom — and the heart of our planet’s sustainable future.
Concluding Observations
Nuclear charge may seem abstract — just a count of protons. But it is a force of nature, shaping the behavior of matter, fueling the stars, and empowering human innovation. From the glow of a distant galaxy to the pulse in your wrist, from the circuitry of your phone to the clean energy of tomorrow — nuclear charge is there, quietly ensuring balance, structure, and life. So next time you look at the periodic table or look at a starlit sky, remember: the heartbeat of every atom is a small, mighty, positive force — building the foundations of a sustainable world.
For more such infomative blogs keep visiting the Atomic Explorersthe, a premier platform dedicated to advancing the understanding of nuclear science and its pivotal role in building a sustainable, energy-efficient world.
Comments